The same forces that separate oil and water in the remaining liquid of a bowl of salad, shape and hold up the cellular structures and, therefore, life. These forces are responsible of the conformation of the biomolecules (which are the chemical compounds that form the living matter) dissolved in the aqueous environment of the cell, depending on if the parts of their structures are attracted, like salt, or repelled, like oil, by the water.
Let’s think about the biomolecule that contains all the information needed to make an organism: deoxyribonucleic acid or DNA. This molecule is similar to charm bracelets which carry pendants every few links. In the case of DNA, the bases that codify the genetic information are hanging in the chain- which is a polymer of sugars and phosphates. Because the bases hate water more severely than oil, they protect themselves by wrapping the chain of sugars and phosphates (as the drinkers of Coca-cola know, these chemical compounds are very soluble in water) around themselves adopting the famous conformation of a double helix.
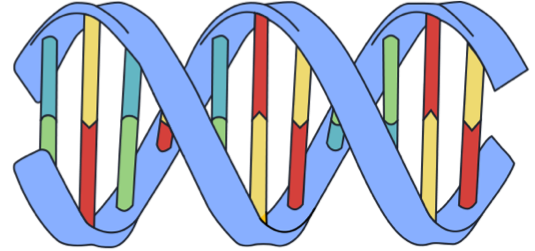
Section of a DNA double helix with the bases protected by the sugar and phosphates chains represented by two blue ribbons.
This way, all our beloved genetic code is very well protected on the inside of the molecule from the toxic products that can be present in the cell that are able to attack the bases and cause mutations and cancer. In fact, the information is so securely stored that the cell itself is unable to read the information unless it first separates the two strands and exposes the bases. This disadvantage turns the search of the points where the replication (copy of the 2 strands to make 2 identical copies of DNA molecule) and the transcription of the genes (reading the recipe to form a protein) start into a task more complicated than finding a needle in a haystack. Moreover, because the cells with longer genomes (basically all the cells that are not bacteria or viruses), save space by wrapping their DNA around barrel-shaped proteins and next, to save even more space, these combinations fold up to produce fibres, the task of searching becomes more complicated that finding a needle in the whole annual Spanish production of hay!
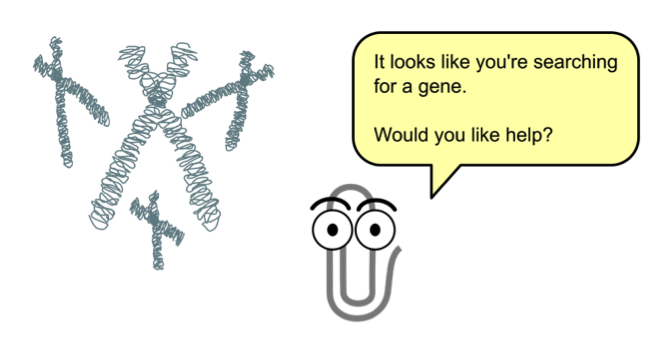
No, Clippy, you can´t help us.
Luckily, the cell has physical and biological mechanisms to find such points, because if not, we wouldn’t be here right now. The biological mechanisms consist of a very complex cellular regulation of all the players that participate in the replication and transcription processes. In the case of the latter, the transcription factors stand out because they are proteins that can recognise the small changes in the shape that the double helix has when the specific sequences that indicate the beginning of a gene appears. Without opening the DNA, these factors signal -to the rest of the involved proteins and enzymes- where they must start their tasks. The complexity of the transcription regulation and the huge variety of transcription factors are the reason why the right genes are expressed at the right time creating a wide variety of plant and animal cells. Only then you will understand why we are so different from a plant, a worm, and mouse when the BBC documentaries say they share significant percentages of their genome with human beings.
The physical mechanisms by which DNA indicates the points where to start copying or reading its message are much less known, mainly because of the difficulty of its study. The basis of these mechanisms is that DNA is not the static entity that appears in our biology book, in the intro of House, or in Jurassic Park, and in the temperature range in which life happens and because of those temperatures, the atoms that form the molecule are vibrating continuously. Some of these vibrations are collective and involve oscillatory movements of groups of atoms together. In the mid-80s, several groups of theoretical scientists demonstrated that DNA could have collective vibrations traveling through the molecule expanding and contracting the space between its bases (stretching and shortening the weak bonds that connect them) as if the DNA molecule was breathing. DNA takes advantage of these vibrations and, at the point where it wants to signal the beginning of a gene, has a sequence of specific bases that alter the stiffness, shape, and the melting temperature of the double helix in the area. Therefore, when one of those waves passes through it, the change of conditions increases the amplitude of the vibration and breaks the weak bonds that connect the two strands, separating them and exposing their bases to the medium. The gap formed between the two strands is called a DNA bubble.
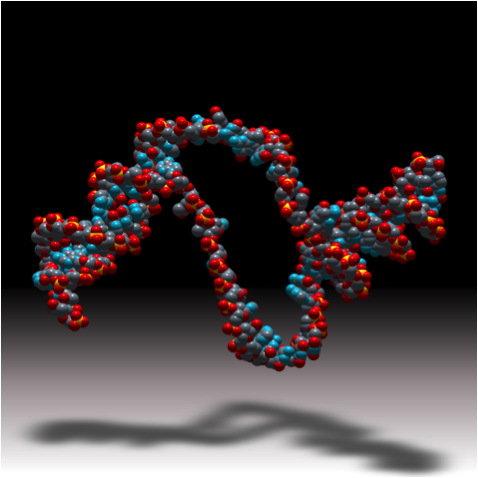
3D model of a DNA double helix with a bubble in the centre.
DNA bubble usually lasts a few little microseconds before returning to the closed configuration due to the tension created in the helix (it is the same kind of tension that appears when someone tries to uncoil the curly cord of a telephone), but at the moment of the replication or the transcription, the enzymes responsible for separating the two strands of DNA, like the helicases, will bind to the bubble, stabilising the open conformation and allowing the attachment to the DNA of the rest of the participant machinery to start its function.
Whereas the bubbles are well known and have even been photographed, the vibrations from which they originate have not been measured until very recently. The reason has been that because they involve the movement of many atoms at the same time, these vibrations have a relatively “low” frequency (they only vibrate around a few billion times per second), which coincides with the frequency of other movements of more small molecules like the rotation of water. As the spectroscopists have to use light of the same frequency than the vibrations in order to study them, they found that the small molecules absorb all the light and prevent seeing the weak collective vibrations of DNA. A solution to the problem was to study samples of dried DNA, although the results were not useful to understand what was happening inside the cell because, as we have seen before, DNA conformation depends on water. Fortunately, in recent years, with the emergence of new technologies, new sensors and new sources of radiation we have been able to begin to address the problem and for the first time, we have been able to measure and characterise the collective vibrations of DNA, increasing our knowledge about its dynamics a little more and giving more importance to the physical mechanisms involved in the biological function of our most important molecule: DNA.
By Dr Mario González Jiménez, Postdoctoral Researcher at the University of Glasgow. SRUK Constituency of Scotland.
For more detailed information, take a look at Mario´s scientific publication: Mario González-Jiménez, Gopakumar Ramakrishnan, Thomas Harwood, Adrian J. Lapthorn, Sharon M. Kelly, Elizabeth M. Ellis y Klaas Wynne. “Observation of coherent delocalized phonon-like modes in DNA under physiological conditions”, Nature Communications. DOI: 10.1038/ncomms11799